Project supported by the National Key Research and Development Program of China (Grant No. 2016YFB0700902), the National Natural Science Foundation of China (Grant Nos. 51590880, 11674379, 51431009, 11674373, and 51625101), and the Youth Innovation Promotion Association of the Chinese Academy of Sciences (Grant No. 2015004).
Project supported by the National Key Research and Development Program of China (Grant No. 2016YFB0700902), the National Natural Science Foundation of China (Grant Nos. 51590880, 11674379, 51431009, 11674373, and 51625101), and the Youth Innovation Promotion Association of the Chinese Academy of Sciences (Grant No. 2015004).
† Corresponding author. E-mail:
Project supported by the National Key Research and Development Program of China (Grant No. 2016YFB0700902), the National Natural Science Foundation of China (Grant Nos. 51590880, 11674379, 51431009, 11674373, and 51625101), and the Youth Innovation Promotion Association of the Chinese Academy of Sciences (Grant No. 2015004).
Lorentz transmission electron microscopy (TEM) is a powerful tool to study the crystal structures and magnetic domain structures in correlation with novel physical properties. Nanometric topological magnetic configurations such as vortices, bubbles, and skyrmions have received enormous attention from the viewpoint of both fundamental science and potential applications in magnetic logic and memory devices, in which understanding the physical properties of magnetic nanodomains is essential. In this review article, several magnetic imaging methods in Lorentz TEM including the Fresnel and Foucault modes, electron holography, and differential phase contrast (DPC) techniques are discussed, where the novel properties of topological magnetic domains are well addressed. In addition, in situ Lorentz TEM demonstrates that the topological domains can be efficiently manipulated by electric currents, magnetic fields, and temperatures, exhibiting novel phenomena under external fields, which advances the development of topological nanodomain-based spintronics.
Lorentz transmission electron microscopy (TEM) has been extensively used to study magnetic domain structures formed due to the interactions between the electron beam and the emergent magnetic fields accompanying their intrinsic local magnetization distribution. It provides detailed insight into magnetic structures as well as compositional and electronic structures with high spatial resolution of 0.1 nm–1.0 nm for structural imaging, 1 nm–3 nm for extraction of compositional information, and 2 nm–20 nm for magnetic imaging.[1–3] The observation of magnetic domains and the corresponding evolution behavior under external fields provides direct link to the macroscopic physical properties and applications.
Formation of magnetic domains is due to the competition among the magnetic energies, such as magneto-anisotropic, ferromagnetic exchange, and magnetostatic energies. The energetically preferred magnetic domains are generated to minimize the total energy.[1,4–7] In recent times, nanoscale topological magnetic configurations have received enormous attention from the viewpoint of both fundamental science and potential application in next generation of spintronic devices with high-density and low-power consumption. Magnetic vortices,[8–10] skyrmions,[6,11,12] and nanometric bubbles[13–15] are typically such topological magnetic configurations. For such magnetic domains with nanometer size, Lorentz TEM with its high spatial magnetic resolution has made significant success in characterizing the detailed spin configurations and the response of those topological magnetic domains to the external magnetic fields,[16,17] temperatures,[18,19] and electric currents,[12,20–22] etc., thus leading to an immense exploration of new materials and potential applications in new spintronic devices.
We give a general introduction of the Lorentz TEM and its application in characterizing the topological spin configurations. In Section
A TEM specimen is characterized under the normal operation mode by using a high-current objective lens (OL) for minimizing the focal length to achieve higher magnification and better spatial resolution. In this case, a strong magnetic field of around 2 T–3 T is applied to the specimen. Such a strong magnetic field saturates the magnetic material, leaving no magnetic domain wall contrast. The intrinsic magnetic domains should be identified under the controllable external fields to provide the real magnetic structure information. In the Lorentz mode, however, we observe the magnetic domains by turning the OL off and manually increasing the magnetic fields continuously by adjusting the OL current in so-called “free lens-control mode” or by using the specially designed JEOL dedicated Lorentz TEM where the residual magnetic field is ignorable at around 4 Oe to 10 Oe (1 Oe = 79.5775 A⋅m−1).[1,23] It should be mentioned that the OL should be switched off during the magnetic sample insertion even for the dedicated Lorentz TEM since the sample is subjected to about 350 Oe field during insertion, which will change the original magnetic domain structures, especially for the soft magnetic materials.[23]
The principle of magnetic domain images obtained from Lorentz TEM or Lorenz mode of standard TEM is based on the electron beam passing through the magnetic material, where the in-plane magnetization induces a Lorentz force normal to both the magnetization and the perpendicular electron beam. The electron beam is deflected by Lorentz force, FL, as a result of the presence of an electrostatic field E and/or a magnetic field B within and around the sample:
![]() |
The most common techniques for imaging the magnetic domains in TEM are Foucault and Fresnel modes, and their corresponding schematics are shown in Fig.
![]() | Fig. 1. (color online) Schematic diagram of ray diagram indicating the paths of electrons passing through a magnetic specimen, together with the magnetic domain or domain wall contrast for the Foucault [(a)–(b)] and Fresnel modes [(c)–(e)] of Lorentz TEM.[27] |
In the Foucault mode as shown in Figs.
For imaging the magnetic domains in Fresnel mode as shown in Figs.
In order to quantitatively determine the direction of magnetization and the spin configuration of magnetic domains using Lorentz TEM images, a method named transport-of-intensity equation (TIE) is generally adopted based on Fresnel mode, which is briefly introduced as follows. When a plane wave of electrons passes through a TEM specimen, the direction of transmitted electrons varies owing to the Lorentz force caused by the interaction between electron wave and specimen, and hence the different image intensity is produced. The image intensity includes both the amplitude and phase information of the transmitted electron wave. TIE can be used to detect the phase information from the intensity distribution as follows:[30]
![]() |
![]() |
![]() |
![]() | Fig. 2. (color online) Schematic intensity distribution of Lorentz TEM images at under-focus, just-focus, and over-focus conditions for TIE method |
Therefore, the lateral magnetization distribution can be quantified using the above TIE method to analyze the electron intensity differences of Lorentz TEM images with different focus values. This knowledge has been developed into a commercial QPt software embedded in widely used Digital Micrograph (DM) TEM interface.
Differential phase contrast (DPC) in Lorentz TEM is a high-resolution magnetic imaging technique for visualizing electromagnetic field distribution via measuring the deflection of an electron beam at each beam-scan point.[28,31,32] The differential phase contrast (DPC) technique, principally described in Fig.
![]() | Fig. 3. (color online) (a) Schematic illustration of the electron beam deflection in a specimen using a segmented STEM detector and top view of the four segments detector presenting the results of a not-deflected beam and deflected beam after passing through the specimen. [(b)–(e)] DPC-STEM experimental observation about magnetic skyrmion in Co8Zn8Mn4.[33] |
Another magnetic imaging technique in Lorentz TEM is off-axis electron holography, which is based on the interference of electron waves passing through the sample (object wave) and the vacuum (reference wave), as shown schematically in Fig.
![]() |
![]() |
The competing magnetic interactions can generate different types of magnetic domains. At the magnetic domain boundary, the magnetic moment continuously transits from one magnetization direction to another, resulting in magnetic domain walls. The typical domain walls are Bloch-type and Néel-type. In the Bloch wall, the magnetic moments gradually rotate in a plane parallel to the wall, whereas in the Néel wall, the magnetic moments rotate in a plane normal to the wall, as shown in Figs.
![]() |
![]() |
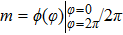
![]() | Fig. 5. (color online) Schematic diagram of spin configurations (a) Bloch wall and (b) Néel wall.[54] Topological magnetic domain structure of (c) vortex,[53] (d) magnetic bubble,[54] and (e) skyrmion.[55] |
The nanometric domains with topological properties have demonstrated strong anti-interference ability and the easily-manipulable behavior, which may lead to their application in magnetic storage and spintronic devices. Uniaxial magnetic anisotropy is an important parameter that determines the topological spin configuration. Magnetic vortex exists especially in submicron disk-shaped nanodots with low uniaxial anisotropy, owing to the edge constriction and the competition between exchange and magnetic dipole–dipole interaction.[56,57] The “in-plane” and “out-of-plane” vortices are schematically shown in Fig.
In parallel with high-resolution Lorentz TEM imaging, in situ manipulation of these topological domains under different external stimulations helps correlate the phase transition and magnetic structures with magnetic properties, prompting these topological magnetic domains into practical applications. Next, we will successively review the generation and manipulation of magnetic vortex, skyrmions, and bubbles, which have been recently studied via Lorentz TEM in the magnetic materials with different anisotropy.
Magnetic vortex has been studied mostly in permalloy with the pattern constriction to exemplify its potential applications in magnetic random access memory.[56,57,60] Lorentz TEM observation gives direct and clear images about the topological magnetic vortices and particularly their dynamic behavior in correlation to the application. Figure
![]() | Fig. 6. (color online) (a)–(c) Lorentz images of the magnetization reversal in the uniformly magnetized domain state at zero magnetic field; (e)–(g) showing the corresponding distribution of magnetic flux lines via holography in panels (b)–(d).[57] |
Besides the typical vortex study in patterned permalloy, amorphous Ce14Fe80B6 ribbon has been recently explored to demonstrate the generation and manipulation of magnetic vortices without pattern constriction.[61] Figure
![]() | Fig. 7. (color online) (a)–(f) Lorentz TEM images for temperature dependence of cross-tie domain wall evolution in amorphous Ce14Fe80B6 ribbon;[61] (c) the enlarged area of red rectangle region of panel (b). The inset of panel (c) shows the corresponding TIE analyzed spin texture of cross-tie wall. Panels (g)–(h) show the schematic spin configuration.[10,62] |
The V/AV propagation and nucleation/annihilation under electric current excitation observed via Lorentz TEM are shown in Fig.
![]() | Fig. 8. (color online) (a)–(h) In situ Lorentz TEM observation for electric current dependence of cross-tie domain wall evolution. (i) Schematic electric-current manipulation device. (j) The summarized number of vortex cores versus current.[61] |
Magnetic skyrmions were originally introduced by the British physicist Tony Skyrme to describe localized, particle-like configurations in the field of pion particles.[63] In recent years, a skyrmion crystal (SkX) with a non-centrosymmetric B20 cubic structure was experimentally identified for the first time in a helimagnet MnSi by small-angle neutron scattering (SANS) measurements. In the ground state, the magnetic structure shows a proper screw structure with a periodicity of ∼ 19 nm, which is induced by Dzyaloshinskii–Moriya interaction (DMI) originating from the symmetry-broken structure. When an external magnetic field is applied, SkX with six-fold symmetry emerges perpendicular to the external magnetic field as shown in Fig.
![]() | Fig. 9. (color online) (a) The skyrmion crystal first identified by SANS measurements in MnSi.[11] (b) and (c) Lorentz TEM observation of skyrmion phase in Fe0.5Co0.5Si[19] and FeGe.[12,66] |
Up to now, numerous magnetic materials hosting skyrmions,[14,66,70] which could be generated via different mechanisms including the dominated symmetry-broken DMI and dipolar interactions, have been discovered. Using Lorentz TEM, biskyrmion spin configuration is identified over a wide temperature range of 16 K–340 K in hexagonal centrosymmetric (Mn1−xNix)65Ga35 (x = 0.5) (MnNiGa) alloy. The biskyrmion evolution from ground stripe domains is clearly demonstrated in Fig.
![]() | Fig. 10. (color online) (a)–(h) Magnetic field dependence of biskyrmions in real-space Lorentz TEM images at room temperature and the corresponding spin texture obtained by TIE analysis.[71] (i) The contour mapping of the external magnetic field and temperature for biskyrmion distribution in MnNiGa extracted from Lorentz TEM data.[18] |
![]() | Fig. 11. (color online) (a) Lorentz TEM image of zero-field biskyrmion lattice at room temperature after FC manipulation. (b) Phase diagram obtained by contour mapping biskyrmion density, as a function of external magnetic field and temperature, based on the Lorentz TEM data after 50-mT FC.[18] |
![]() | Fig. 12. (color online) (a)–(d) Magnetic domain dependence on temperature at 50 mT (e)–(h) Biskyrmion density dependence on the different magnetic fields while FC process. (i)–(l) Biskyrmion distribution after increasing the magnetic field to complete skyrmion state based on panels (e)–(h). The experimental procedures are shown on top of the column. The scale bars are 200 nm.[18] |
Tremendous progress about exploring new skyrmion materials has been made in the multilayer thin film since the discovery of the blowing skyrmion behavior via electric currents in a sandwiched Ta/CoFeB/TaO multilayer with interfacial DMI.[73] Room temperature skyrmions, with their electric field-driven behavior and compatibility with current spintronic fabrication techniques in thin films can be readily integrated into the spintronic devices. The skyrmion characterization and dynamic behavior can be studied using various instruments. For example, writing and deleting a single skyrmion is studied using spin-polarized tunneling currents at low temperatures using spin-polarized scanning tunneling microscopy (SP-STM).[74] Blowing out room-temperature skyrmions due to electrical currents are observed using skyrmion Hall effect by magneto-optical Kerr effect (MOKE).[73,75] Artificial skyrmions are detected due to the interlayer coupling in thin films by scanning electron microscopy with polarization analysis (SEMPA).[76]
Lorentz TEM has advantages in demonstrating the spin configuration and dynamic behavior of nanometric magnetic domains under different external fields. The spin configuration of typical Néel-type skyrmions in multilayers can be identified by tilting the specimen based on the magnetic field-induced beam deflection and the contrast mechanism of Lorentz TEM, which can be summarized as the disappearance of contrast at a position and reversal of contrast at relatively opposite angles.[68,77] Moreover, the multilayers sputtered on the Si3N4 membrane windows for direct TEM observation avoid the extrinsic features introduced by additional TEM preparation. Néel-type magnetic skyrmions were successfully identified in Ta(4)/[Pt(3)/Co(1.85)/Ta(3)]6 multilayers based on their response to opposite tilting angle of 18° as shown in Figs.
![]() | Fig. 13. (color online) Identification of skyrmion spin configuration by tilting samples in real-space Lorentz TEM at 300 K. (a) Schematic diagram of titling process, α is the tilt angle around x-axis. The angle α for Pt/Co(1.85)/Ta is (b) −18°, (c) 0°, and (d) 18°, respectively. The enlarged views of a single skyrmion in the insets (b) and (d) with bright and dark reversed magnetic contrast for the opposite tilting angle are identified as Néel-type skyrmion. The tilting angle α are (e) −18°, (f) 0°, and (g) 18° for Pt/Co(1.95)/Ta, respectively. The tilting angle α are (h) −20°, (i) 0°, and (j) 20° for Pt/Co(2.1)/Ta, respectively. Scale bars in panels (b)–(j) correspond to 50 nm.[78] |
![]() | Fig. 14. (color online) The Lorentz TEM image of the Néel-type skyrmions in Ta(4)/[Pt(3)/Co(1.85)/Ta(3)]6 multilayers, demonstrating the comparison between the nonvolatile field-free skyrmions after electromagnetical manipulation and the randomly distributed biskyrmions induced purely by the magnetic field.[79] |
Figure
![]() | Fig. 15. (color online) Magnetic skyrmion evolution by tuning the electric current density, at a fixed magnetic field of 270 Oe, at values: (a) 0, (b) 5.01, (c) 6.81, (d) 7.36 (× 108 A⋅m−2). (e) High-density skyrmions at J = 0. (f) Zero-field and high-density skyrmions at J = 0 and H = 0. The scale bar in panel (a) is 200 nm.[79] |
Electron spins play a critical role and contribute differently in functional magnetic materials. In 2008, Parkin et al. proposed that the polarization current drives the domain wall movement via spin transfer torque effect, thereby realizing the information reading and writing in the racetrack memory.[80] From the aspect of device application, dynamic current-driven motion of magnetic skyrmion is superior to the normal domain wall due to the lower current density requirements. The interactions between the traversed electrons and the topological skyrmions give rise to the topological Hall effect. As a counteraction of the topological Hall effect related to the electron Hall motion, the skyrmion or SkX itself exhibits a Hall motion.[6] Similar to the FM domain wall motion driven by spin-polarized electric current, the flowing electric current drives the skyrmions not only along the electron flow direction but also along the transverse (Hall) motion as shown in Figs.
Traditional magnetic bubble domains were studied as early as 1970 with the corresponding information storage device commercialized at that time.[81,82] With the development of high-resolution techniques, the detailed spin configuration inside the domain wall and the discovery of nanometer bubbles led to a new surge of research interests. The topological states of traditional magnetic bubbles are usually various and random, as shown in Fig.
![]() | Fig. 17. (color online) (a)–(d) Magnetization dynamics through Bloch line (BL) motion induced by the in-plane magnetic fields. (e)–(h) Analyzed spin configurations for the bubbles shown in panels (a)–(d), respectively. (g)–(i) Temporal evolution of magnetization accompanied by annihilation of BLs.[14] |
Despite the complex spin configuration in the above-mentioned narrow domain wall region, the domain morphology favors the inside magnetization direction along the easy-axis, which usually requires the quality factor Q (Q = Ku/Kd = μ0Hk/μ0Ms) larger than 1. The enhancement of quality factor Q caused by the substitution of rare-earth element Y in MnNiGa contributes to the transition from biskyrmions to bubbles as shown in Fig.
![]() | Fig. 18. (color online) Topological transition from (a) biskyrmion configuration in MnNiGa[71] to (b) magnetic bubbles with various topological states in MnNiGaY by substitution of rare-earth element Y. (c) M–H curve, for the oriented and isotropic bulk samples, measured parallel and perpendicular to the direction of easy magnetization, and the inset showing the second derivative (d2M/dH2) curves of perpendicular magnetization and the M–1/H curve.[83] |
To conclude, Lorentz TEM as a magnetic imaging instrument is a promising tool to characterize the topological magnetic structures with a high magnetic spatial resolution. Several operated methods including the Fresnel and Foucault modes, electron holography, and DPC techniques can be brought to use. Different functional TEM holders broaden the scope of in situ experiments to be performed under varied external fields, electric current, and temperature fields. The real-space observation of topological magnetic domains such as magnetic vortex, skyrmions, and bubbles under the multi-fields manipulation in Lorentz TEM reveals the generation mechanism and the correlation with physical properties, which can promote the application of topological materials in the information storage field and lead to the exploration of new topological materials.
[1] | |
[2] | |
[3] | |
[4] | |
[5] | |
[6] | |
[7] | |
[8] | |
[9] | |
[10] | |
[11] | |
[12] | |
[13] | |
[14] | |
[15] | |
[16] | |
[17] | |
[18] | |
[19] | |
[20] | |
[21] | |
[22] | |
[23] | |
[24] | |
[25] | |
[26] | |
[27] | |
[28] | |
[29] | |
[30] | |
[31] | |
[32] | |
[33] | |
[34] | |
[35] | |
[36] | |
[37] | |
[38] | |
[39] | |
[40] | |
[41] | |
[42] | |
[43] | |
[44] | |
[45] | |
[46] | |
[47] | |
[48] | |
[49] | |
[50] | |
[51] | |
[52] | |
[53] | |
[54] | |
[55] | |
[56] | |
[57] | |
[58] | |
[59] | |
[60] | |
[61] | |
[62] | |
[63] | |
[64] | |
[65] | |
[66] | |
[67] | |
[68] | |
[69] | |
[70] | |
[71] | |
[72] | |
[73] | |
[74] | |
[75] | |
[76] | |
[77] | |
[78] | |
[79] | |
[80] | |
[81] | |
[82] | |
[83] |