† Corresponding author. E-mail:
Project supported by the National Key Research and Development Program of China (Grant No. 2017YFA0701000), the National Key Scientific Instrument and Equipment Development of China (Grant No. 2018YFF01013001), and the National Natural Science Foundation of China (Grant Nos. 61701084 and 61505022).
We demonstrate a physical mechanism for terahertz (THz) generation from surface plasmon polaritons (SPPs). In a structure with a bulk Dirac semimetals (BDSs) film deposited on a dielectric substrate, the energy of the asymmetric SPP mode can be significantly enhanced to cross the light line of the substrate due to the SPP-coupling between the interfaces of the film. Therefore, the SPPs can be immediately transformed into Cherenkov radiation without removing the wavevector mismatch. Additionally, the symmetric SPP mode can also be dramatically lifted to cross the substrate light line when a buffer layer with low permittivity relative to the substrate is introduced. In this case, dual-frequency THz radiation from the two SPP modes can be generated simultaneously. The radiation intensity is significantly enhanced by over two orders due to the field enhancement of the SPPs. The radiation frequency can be tuned in the THz frequency regime by adjusting the beam energy and the chemical potential of the BDSs. Our results could find potential applications in developing room temperature, tunable, coherent, and intense THz radiation sources to cover the entire THz band.
Great attention has been paid to three-dimensional (3D) Dirac semimetals, also called bulk Dirac semimetals (BDSs), due to the recent experimental discovery of Cd3As2,[1–3] Na3Bi,[4] and ZrTe5.[5] As confirmed by angle-resolved photoemission spectroscopy, BDSs are characterized by a linear dispersion relationship for fermion quasiparticles along all three momentum directions, and thus they can be viewed as 3D counterparts to graphene. Graphene has demonstrated to have great potential in the application of subwavelength plasmonic devices.[6–11] Surface plasmon polaritons (SPPs) that are supported on graphene have the properties of extremely high confinement and low Ohmic loss because of their large carrier mobility.[6,12,13] As types of 3D graphene, BDSs can also sustain SPPs in the terahertz (THz) to mid-infrared regimes. The much higher mobility of BDSs, arising from the crystalline symmetry protection against gap formation,[14] may lead to advances in SPPs properties. Additionally, an extra dimension brings essential differences to some property of the SPPs, i.e., the SPP-coupling between the interfaces of the BDS film results in the splitting of the SPP dispersion into symmetric and asymmetric modes, and the characteristics of SPPs are influenced by the thickness of the BDS film.[15] Recently, intense study of the plasmon mode arising in BDSs has been carried out,[16–20] and some unique features have been uncovered.[16,17]
In the past few decades, THz radiation has become the most attractive research area in modern science and technology due to its unique characteristics and wide potential applications.[21–24] However, the development of room temperature, miniature, high powered and tunable THz radiation sources covering the entire THz frequency regime remains a significant challenge. Recently, free-electrons driven graphene plasmonics have provided exciting opportunities for THz radiation sources.[25–31] The desired THz radiation can be developed mainly depending on the remarkable properties of graphene SPPs. The well-known wavevector mismatch between SPPs and electromagnetic waves in free-space prevents direct SPP-radiation coupling. To compensate for this momentum mismatch, particular effects are needed, such as periodic zone folding,[25–27] nonlinearity,[28] circular periodicity,[29] sidewall,[30] and buffer layer effects.[31] In these works, the diffraction radiation or Cherenkov radiation mechanisms were involved. The radiation intensity of the Cherenkov effect was two to three orders of magnitude higher than that of diffraction radiation.[29,31]
In this article, we present a physical mechanism in which coherent and tunable THz radiation can be generated from SPPs excited by a moving electron beam on top of a BDS film deposited on a dielectric substrate. Due to the SPP-coupling between the interfaces of the film, the SPP dispersion splits into symmetric and asymmetric modes, and the energy of the asymmetric SPP mode is greatly enhanced to cross the light line of the substrate, thus, making the transformation of the SPPs into radiation immediately possible. Two-color THz radiation can be generated from symmetric and asymmetric SPP modes when a buffer layer with a lower permittivity relative to the substrate is introduced. Compared to the radiation without SPPs, the radiation intensity is enhanced by SPPs by over two orders up to 6.7 × 104 W/cm2. The radiation frequency can be tuned in the THz frequency band by varying the energy of the electron beam and the chemical potential of the BDSs.
We first considered the SPPs excitation by an electron beam moving on top of a BDS film suspended in vacuum, as shown in the inset of Fig.

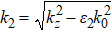
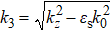

Based on the dispersion Eqs. (
We have demonstrated the efficient excitation of SPPs in BDS film by an electron beam. Now we will show the transformation of SPP into THz radiation in a structure of BDS film with a dielectric substrate loading, as depicted in Fig.
The symmetric SPP mode could not be transformed into radiation because its dispersion curve always lay below the light line in the dielectric substrate. We added a dielectric buffer layer with a thickness h between the BDS film and the substrate, as shown in Fig.


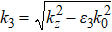
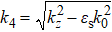
The dispersion curves of the structure with a buffer layer based on the dispersion Eq. (
The radiation intensity was significantly enhanced by the excited SPPs. It should be pointed out that, without the excitation of the SPPs, Cherenkov radiation could also occur. It was transformed from the evanescent wave generated by the electron beam when the wave-phase velocity was greater than that of the light in the substrate. For the symmetric SPP mode, the enhancement was greater by a factor of 263. The underlying physics of the large enhancement meant that the intensity of the excited SPPs was two or three orders higher than that of the evanescent wave. The power density could be evaluated by the method proposed in Refs. [26,31] The radiation power density was proportional to the square of the charge density of the electron beam.[31] For a charge density of 100 pC/cm, the peak radiation power density could reach 6.7 × 104 W/cm2. This was comparable to the radiation from graphene SPPs.
The experimental work demonstrated the stability of the bulk Dirac fermion since the chemical potential was tuned by surface doping.[4] The dependence of the radiation frequency on the chemical potential and beam energy is shown in Fig.
We presented a physical mechanism of THz generation from SPPs in the structures of BDS film deposited on a dielectric substrate with or without a buffer layer. We found that the asymmetric SPP mode could cross the light line of the substrate due to the dramatically increased energy of the SPPs caused by the splitting of the SPP dispersion. The dispersion of the symmetric SPP mode could also be lifted to cross the light line in the substrate by a buffer layer with a lower permittivity relative to the substrate. Based on these mechanisms, one-color or two-color THz radiation from SPPs excited by a moving electron beam atop BDS film was analyzed. The results showed that the radiation intensity was significantly enhanced over 200 times up to 6.7 × 104 W/cm2. Furthermore, the radiation frequency could be tuned in the THz frequency regime by adjusting the beam energy or the chemical potential of the BDSs. Based on this mechanism, the room temperature, tunable, coherent, and intense THz radiation sources could be developed.
[1] | |
[2] | |
[3] | |
[4] | |
[5] | |
[6] | |
[7] | |
[8] | |
[9] | |
[10] | |
[11] | |
[12] | |
[13] | |
[14] | |
[15] | |
[16] | |
[17] | |
[18] | |
[19] | |
[20] | |
[21] | |
[22] | |
[23] | |
[24] | |
[25] | |
[26] | |
[27] | |
[28] | |
[29] | |
[30] | |
[31] |