1. IntroductionIn the past 20 years, dye-sensitized solar cells (DSSCs) were intensively investigated by researchers and industries owing to their low cost, easy fabrication and high photoelectric conversion efficiency (PCE).[1–5]
DSSC consists of a wide-bandgap nanocrystal semiconductor anode, an electrolyte and a counter electrode.[6,7] In order to realize a high PCE, wide-bandgap semiconductors must satisfy the following requirements: (i) bandgap is larger than 3.2 eV to reduce the adsorption of visible light;[8] (ii) conduction band edge needs to align with the lowest unoccupied molecular orbital (LUMO) of the dye; (iii) carrier has the fast transport rate; (iv) the rate of recombination between electron in semiconductor and electrolyte is slow. Up to now, few materials can satisfy both requirements (iii) and (iv). TiO2 has a slow electron recombination rate and a slow electron transport rate. On the contrary, ZnO[9–14] and SnO2[15–19] have fast electron transport rates and a fast electron recombination rate. Among all the semiconductors, DSSCs with TiO2 as anode have the highest PCE, larger than those with of ZnO and SnO2. However, the electron transport rate in TiO2 is lower than that of ZnO and SnO2. In order to increase PCE of DSSCs, much research has been carried out on the understanding which makes the electron transport rate slow and how to improve the electron transport rate of TiO2.
The transient current and intensity-modulated photocurrent spectroscopy (IMPS) are used to investigate electron transport properties in semiconductor nanocrystalline film. It is found that the electron transport rate is 1–3 orders of magnitude slower than that of bulk materials and proportional to the square root of electron density. Most popular trap–detrap models can explain these results. Electrons are trapped by defect states during the transport, and the trapped electrons need to be excited by heat to detrap to the conduction band to transport again. With the increase in electron density, the occupation of trapped states is increased, which makes rate of electron trap slower and detrap faster, and consequently the transport rate is increased. Moreover, the disordered distribution of nanoparticles increases the transport path length, then decreases the transport rate.
The core–shell structure is one of the possible methods to enhance electron transport rate. ZnO or SnO2 nanoparticles covered by TiO2 may combine the advantages of the high electron transport rate of the core and the low electron recombination rate of the shell. However, the result of the core–shell structure is not as good as we expected. This is because the energy level of the shell does not match with the core and the poor electronic contact between the core and shell, or the recombination between core and electrolyte is not fully inhibited by the shell owing to poor morphology.
In this paper, we designed and fabricated the photoanode of DSSCs, which centered on an Al2O3 core surrounded by a TiO2 shell (A/T(n)). We used this core–shell structure as a model to investigate the effect of electron transport rate on photoelectric properties of cells.
and PCE peaked at A/T(4), with values of
and 3.32% respectively. This study provides a method to study the mechanism of electron transport in nanocrystalline porous film.
2. Experimental section2.1. Preparation of TiO2 compact layerIsopropyl titanium (0.455 mL) was added to n-propanol (20 mL) to form a TiO2 paste under stirring for 12 h at room temperature. The TiO2 compact layer was prepared on the FTO plate using spin-coating technique, and then was heated at 450 °C for 30 min.
2.2. Preparation of A/T(n) photoanodeAl2O3 (0.4 g), triton-100 (0.2 g), acetic acid (0.4 ml), and deionized water (2 ml) were first mixed to form the Al2O3 paste. The Al2O3 paste was ultrasonically dispersed for 2 h first and then stirred for 24 h. The Al2O3 films were fabricated on the TiO2 compact layer using a doctor blade technique, and then were heated at 450 °C for 30 min. The annealed Al2O3 films were immersed into an aqueous solution of TiCl4 (0.07 M) for post-treatment at 70 °C for 30 min.[20,21] Then, the Al2O3 films were flushed with deionized water, dried and annealed again at 450 °C for 30 min to form the A/T photoanode. The thickness of TiO2 from A/T(n) photoanode could be adjusted by repeating cycles of the TiCl4 treatment.
2.3. Fabrication of DSSCsTo eliminate the physisorbed water, the A/T photoanode was heated at 100 °C for 40 min and then immersed in ethanol solution of the N3 dye of 0.3 mM for 24 h. The dye-sensitized A/T photoanode together with a platinum foil counter electrode and liquid electrolyte composed of 0.5 M LiI, 0.02 M I2, 0.3 M 1-methyl-3-hexylimidazolium iodide (HMII),[22] and 0.5 M 4-tert-butylpyridine in 3-methoxypropionitrile were assembled into a sandwich cell structure.
2.4. Characterization and measurementsThe crystallographic structure of the A/T film was characterized by x-ray diffraction (XRD) (Rigaku D/max-2500) Cu Kα radiation with a scan speed of 8°/min. Surface morphology studies were carried out by scanning electron microscopy (SEM, Hitachi S-4800, 15 kV) and high-resolution transmission electron microscopy (HR-TEM, Tecnai G2 20 S-TWIN, 200 kV). The electrochemical performance was investigated by a Keithley 2611 Source Meter (Keithley Instruments, Inc., USA) under AM 1.5 (
) supplied by a solar simulator (Oriel, 91160–1000). Electrochemical impedance spectroscopy (EIS) data were obtained under
illuminations, using a perturbation of ±10 mV over the open-circuit potential by using Solartron 1255B frequency analyzer and Solartron SI 1287 electrochemical interface system. The intensity-modulated photocurrent and photovoltage spectroscopy (IMPS and IMVS) were performed using a green light emitting diode (max = 520 nm) driven by a solartron 1255B frequency-response analyzer. The LED provided both the dc and ac components of the illumination. The UV spectrum measurement was made with aU-3010 spectrophotometer (Hitachi Corp).
3. Results and discussion3.1. XRD analysisFigure 1 shows the XRD patterns of FTO, Al2O3 film, and A/T(4) film. It can be seen that the diffraction peaks of 25.64°, 35.18°, 43.5°, 52.66°, 57.68°, 66.56° can match well with the standard rhombohedron phase of Al2O3 (JCPDF No. 46-1212). Compared to the Al2O3 film, the diffraction peaks of 25.34°, 53.49°, 68.3°, 69.1° from A/T(4) film can be assigned to standard anatase phase of TiO2 (JCPDF No. 21-1272). It is evident that TiO2 particles can form on the Al2O3 particles by TiCl4 post-treatment. The slight decrease in peak strength of Al2O3 may be due to the Al2O3 layer coated by the TiO2 layer from TiCl4 hydrolysis, which weakens the relative strength.
3.2. Morphology of A/T(n)The Morphology of the Al2O3 film and the A/T(n) film is investigated by SEM and TEM (Figs. 2 and 3). It can be seen that the surface of the Al2O3 film is relatively rough compared to the A/T(4) film. TiO2 particles formed by TiCl4 hydrolysis grow on the Al2O3 particles, which make the surface of Al2O3 film smooth. The diameter of Al2O3 particles measured from the TEM image (shown in Fig. 3(a1)) is approximately 80 nm. As the time of TiCl4 treatment increases, so does the number of TiO2 particles and the diameter of TiO2 is approximately 10–20 nm.
3.3. Photoelectric conversion propertiesDye adsorption increases from
of A/T(1) to
of A/T(4) only improve 50%. The Al2O3 films without TiCl4 treatment also can adsorb dye on the Al2O3 particles with the value of
. Though Al2O3 can adsorb dye, Al2O3 cannot generate photocurrent because of its insulating properties. The increased surface area of the new TiO2 particles formed on the Al2O3 surface is mostly canceled out by the overlap of TiO2 on Al2O3 or overlap between TiO2 particles. Dye adsorption falls to
for A/T(5) because the TiO2 particles may block the channel and prevent the solution of dye connecting with the inner TiO2 particles. The
of A/T(1) is nearly zero, although the dye adsorption is quite large. The electrons neither inject into Al2O3 from adsorbed dye nor transfer in the network of Al2O3 to form photocurrent. The electrons can inject into TiO2 from adsorbed dye but cannot transfer from one TiO2 particles to another because of the isolation of TiO2 particles. With the increase in TiCl4 treatment times, the number of TiO2 particles increases and, consequently, the particles are close to each other and form a conducting network. Electrons can transport in the network. Moreover, the increase in the number of TiO2 particles formed on the surface of Al2O3 particles increases the amount of TiO2 absorbing dye and reduces the amount of Al2O3 absorbing dye, which results in
increasing remarkably to
with the increase in the TiCl4 treatment times up to 4. However,
is only 5.61 for A/T(5), owing to low dye adsorption and block of the contact between the dye and solution.
The
of DSSC of A/T(n) has opposite trends compared to
with the increase of n. The
has the largest value of 745 mV for n = 1 and decreases with increasing n. The
of A/T(4) is the smallest and only 655 mV. If we increase n to 5, the
of A/T(n) increases again. Generally, the
is determined by the difference of conduction and redox potential of the electrolyte, and the electron recombination rate. The dye absorption may represent the surface area of TiO2. From the dye absorption data, we know that A/T(4) has largest surface area. The larger the surface area is, the higher recombination rate is. Thus, the
and
of A/T(n) display opposite behavior with increase in n.
The FF of A/T(n) reduces with the increase in n. The low value of A/T(1) may be due to the large error caused by the low value of
.
The
of A/T(4) is higher than all of other treatment times, although
and FF of A/T(4) are slightly lower than all of other treatment times. We obtained the highest PCE of 3.32% of A/T(4).
3.4. IMPS and IMVSIMPS and IMVS of A/T(n) (n = 1–5) are shown in Fig. 5. Electron transport time (
) in A/T(n) is calculated from the frequency of the lowest point in the IMPS plot and listed in Table 2.
represents average time of electrons transport from injecting into TiO2 to reaching the external circuit. The smaller the value of
is, the faster the electron transfer rate is. The highest
is in accordance with the shortest
(2.02 ms) obtained from A/T(4). However, the amplitude of
change is less obvious than the
change from A/T(1) to A/T(5) (Tables 1 and 2). Hence, the rise of
is only partly due to the increase of electron transfer rate. The formation of the conducting network of TiO2 particles may also affect the
. From the TEM images, it can be seen that TiO2 particles become more and more and closely linked to constitute a conducting network with the increase times of TiCl4 treatment. The expanding scope of conducting network causes the significant rise in the
from A/T(1) to A/T(4). At the same time, the increasing TiO2 particles in the conducting network can also enhance
. Because TiO2 particles connect to each other closely to form more routes for electron transport that make the electrons injected into the TiO2 particles reach the substrate easier. Therefore, the electron transport in the network can choose a short path directly to the substrate and we get a very short
in A/T(4). For A/T(5), TiO2 particles may block the channel of electrolyte, and the ion in the electrolyte cannot transport alone with electrons to keep the electric neutrality. The movement of an electron would form an electrical field against the transport of electrons, resulting in the decrease in
.
Table 1.
Table 1.
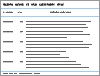 | Table 1.
Photoelectric conversion parameters of A/T(n).
. |
Table 2.
Table 2.
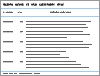 Table 2.
Electron transportation time and lifetime.
.
Sample |
 |
 |
τt/ms |
τn/ms |
A/T(1) |
34.033 |
0.229 |
6.64 |
815.44 |
A/T(2) |
35.397 |
0.891 |
4.5 |
178.67 |
A/T(3) |
42.14 |
0.398 |
3.79 |
399.97 |
A/T(4) |
79.771 |
0.336 |
2.02 |
555.31 |
A/T(5) |
50.332 |
0.22 |
3.21 |
723.8 |
| Table 2.
Electron transportation time and lifetime.
. |
For A/T(1) or A/T(2), the conducting network is sparse, and electrons must be transported over a long curved path to reach the electrode, which increases
. However, TiO2 particles on the top of electrode are difficult to connect to the network, and most of TiO2 particles in the network are close to the FTO electrode. Therefore,
of A/T(1) or A/T(2) is less than 3 times of that of A/T(4).
Generally, electron transport in nanocrystalline is slower than in bulk materials. Compared with pure TiO2 nanocrystalline film, TiO2 particle in A/T(n) is smaller, and the transport length is longer owing to the Al2O3 core blocking the conducting path. However, the
of A/T(n) is the same magnitude as pure TiO2 films. The volume of TiO2 particles is smaller than that of pure TiO2 films, and the electron density in TiO2 of A/T(n) is higher. High electron density may fill the trap state and accelerate the electron transfer rate.
The electron lifetime τn in A/T(n) is also calculated from the frequency of the lowest point in IMVS plot and listed in Table 2. τn represents the average time of electrons staying in TiO2 particles before they recombine with electrolyte or oxidized dye. Generally, longer τn means larger
. However, this relation is not clear in our experiments. A/T(2) has shortest electron lifetime, but its
is not the smallest, and only slightly smaller than A/T(1). We think that the IMVS measurement may has some error on A/T(2) and A/T(3).
3.5. EISThe Nyquist plot of A/T(1–5) is shown in Fig. 6. In the Nyquist plot, EIS is composed of two semicircles. The interception of the high-frequency semicircle with the X-axis represents the serial resistance (
of the cell, which consists of the resistance of electrolyte, FTO film, and TiO2 working electrode. TiCl4 treatment does not affect the resistance of electrolyte and FTO film. The variation of
with treatment times is due to the change in the resistance of working electrode, because the increased number of TiO2 particles provides a better conducting network and reduces the serial resistance of the working electrode. The high-frequency semicircle represents the impedance of the counter electrode, which has nothing to do with the working electrode investigated in this study, and has not been analyzed. The low-frequency semicircle represents the impedance of recombination on the interface of TiO2 and the electrode. The increased number of TiO2 particles has larger surface area, but may also block the electrolyte channel. Those two effects lead the
to first decrease and then increase after four times treatment.
Table 3.
Table 3.
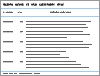 Table 3.
Serial and recombination resistance of A/T(n).
.
Sample |
 |
 |
A/T(1) |
44.31 |
229.1 |
A/T(2) |
38.83 |
51.98 |
A/T(3) |
34.3 |
26.46 |
A/T(4) |
24.81 |
29.37 |
A/T(5) |
16.13 |
27.03 |
| Table 3.
Serial and recombination resistance of A/T(n).
. |
4. Conclusion and perspectivesTiO2 nanoparticles were formed by TiCl4 treatment on the framework of insulating Al2O3 nanoparticles of diameter 80 nm. The influence of various behaviors, such as photoelectrical, morphology, electron transport, and recombination, on the treatment times was investigated. With the increase in treatment times, the number of particles rather than diameter of particles were increased.
and PCE reached peak at A/T(4) of
and 3.32% respectively. Electron transport time
of A/T(n) obtained from IMPS measurement showed that TiCl4 treatment times only slightly affected the
, and its value was the same amplitude as pure TiO2. Combining with TEM images of A/T(n), we thought that with the increase in n, the range and the inner connection of the conduction network was increased. The former increased the
, and the later decreased the
and serial resistance
. A/T(n) film provides a new model to understand the insight of electron transportation in semiconducting nanoparticles.