† Corresponding author. E-mail:
Project supported by the National Natural Science Foundation of China (Grant Nos. 61474104 and 61504131).
Te-doped GaSb single crystals are studied by measuring Hall effect, infrared (IR) transmission and photoluminescence (PL) spectra. It is found that the n-type GaSb with IR transmittance can be obtained as high as 60% by the critical control of the Te-doping concentration and electrical compensation. The concentration of the native acceptor-associated defects is apparently low in the Te-doped GaSb compared with those in undoped and heavily Te-doped GaSb. The mechanism for the high IR transmittance is analyzed by considering the defect-involved optical absorption process.
Gallium antimonide (GaSb) is an important III–V semiconductor material used for manufacturing infrared detectors, laser diodes, high-frequency devices, superlattices and LEDs.[1–3] Recently, one of its most important applications is to serve as a substrate material for infrared detectors such as type-II superlattices InAs/InAsSb,InAs/GaSb,[4,5] especially in the focal plane array (FPA).[6,7] The third-generation infrared FPAs have extensive applications in both military and civilian fields, such as infrared night vision system, object identification and atmosphere surveillance. However, there exists very strong absorption in the GaSb substrate under back-side illumination configuration when the substrate is not completely removed in fabricating quad FPA, thus it is necessary to reduce the absorption of GaSb substrate and enhance its below-band gap transmission. For this purpose, the IR transmission of GaSb should be as high as possible. Unfortunately, as-grown GaSb usually exhibits p-type conduction caused by native gallium vacancies (VGa) and gallium anti-sites (GaSb) at a concentration of 1017 cm−3.[8,9] The VGa and GaSb defects serve as doubly ionizable native acceptors,[10,11] generating strong below-band gap absorption and electrical compensation of n-type doping.[12]
In principal, the main absorption mechanism of GaSb is inter-valence band absorption based on the transitions from the light-hole to the heavy-hole band in the p-type GaSb, while free-carriers, inter-valley conduction band electron absorption mechanism dominates in n-type GaSb.[13,14] Therefore, GaSb wafer with high transmission can be obtained through a proper Te doping compensation which not only compensates for the vacancies and native acceptors but also keeps carrier absorption as low as possible. Liquid encapsulated Czochralski (LEC) technique[15] is a conventional crystal growth method which is suitable for the mass-production of high quality GaSb single crystal. Its drawback is that a high concentration of native acceptor defect is easily formed in the growth process. In this paper, we focus on the enhancement of IR transmission of GaSb substrate material obtained from LEC growth method with tellurium doping compensation. Optical property of Tedoped GaSb has been studied by several groups by using crystal samples grown by horizontal Bridgman (HB),[16–19] solution growth method,[20,21] etc. In this paper, various lightly Te-doped n-type LEC-GaSb samples are used to study the associated mechanism and obtain high transparency substrates through controlling the doping concentrations and compensating for the native acceptor defects.
Te-doped GaSb bulk single crystal ingots with (100) orientation were grown by LEC technique. The (100) wafers each with 750-μm thickness were sliced and polished on both sides for measuring the infrared optical properties. The electrical properties, such as carrier concentration, mobility and doping condition were investigated through a Hall measurements system at room temperature. The IR transmittances of the samples were measured by an FTS-60V Fourier transform infrared spectrometer system (FTIR). Defects in the samples were investigated by PL spectra obtained at 77 K by using a Bruker Vertex 80 FTIR equipped with an InSb detector. The excitation power of the PL spectra was 100 mW.
In order to obtain GaSb single crystal with good below-band gap transmission, the relationship between doping concentration, defect concentration and IR transmittance needs to be identified. The transmittance and absorption coefficient can be obtained through the following formula:[13]
![]() |
![]() |
![]() |
![]() |
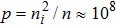
![]() |
![]() |
![]() |
In the present work, Te-doped GaSb samples are chosen for the investigation in order to obtain the IR transmission as high as possible. As has been mentioned, the main absorption mechanisms are inter-valley conduction band absorption and the free electron absorption in the n-type GaSb. From the formula, we know that the main absorption mechanism is inter-valley conduction band absorption in a range from 2.5 μm to 5 μm, there is barely no absorption for inter-valley conduction band absorption after 15.5 μm and the only absorption mechanism is electron free carrier absorption. For the Te-doped n-type GaSb sample, the concentrations of residual holes, vacancies and native acceptor defects fall and the transmission increases because of the Te doping compensation. However, when the Te content increases too much (heavily Te-doped), beyond 1 × 1017 cm−3 for instance, the free carrier absorption becomes the main constrained element and the absorption increases promptly due to the excessive free carriers, resulting in a rapid transmission drop. Therefore there should be an appropriate Te doping content that not only compensates for the residual holes, vacancies and native acceptor defects but also generates suitable free carrier concentrations to keep the minimum absorption. Electrical properties of GaSb samples with different Te doping content are shown in Table
![]() | Table 1.
Hall results of n-type Te-GaSb samples at room temperature. . |
Figure
From Eq. (
Figure
Vlasov et al.[11] proved that the full width at half maximum (FWHM) of peak C is related to native defect concentration and increases with defect concentration increasing. Figure
Te-doped GaSb single crystal with a carrier concentration of 1016 cm−3 and good mobility has below-band gap transmittance as high as 60%. The high transmittance is ascribed to low absorption relating to a very low native acceptor concentration and weak free carrier absorption due to the low carrier concentration.
[1] | |
[2] | |
[3] | |
[4] | |
[5] | |
[6] | |
[7] | |
[8] | |
[9] | |
[10] | |
[11] | |
[12] | |
[13] | |
[14] | |
[15] | |
[16] | |
[17] | |
[18] | |
[19] | |
[20] | |
[21] | |
[22] | |
[23] | |
[24] | |
[25] | |
[26] | |
[27] | |
[28] | |
[29] | |
[30] |